Advanced Nuclear Learns to Share the Dance Floor With Renewables
Variable renewable energy means “baseload” alone won’t cut it
By Matthew L. Wald
Among the disconnects between energy engineers and policymakers is on the term “baseload.” It’s a shame, because a better understanding would mean a better appreciation of the benefits of advanced nuclear, and the grid’s requirement for clean, firm, flexible generation.
Today’s reactors don’t have to operate flexibly, or, at least, they didn’t until recently. They meet a part of the demand that is called “baseload,” meaning the minimum amount needed to meet the lowest level of customer demand on a typical day.
Today, baseload plants run flat out, and plants that meet variable demand will power up and power down over the course of the day.
To cite an example, the independent system operator that runs the grid in the six-state New England region reported that for June 12 of this year, demand varied from about 10,400 megawatts to about 15,200 megawatts. (For comparison, a big nuclear plant can make a little over 1,000 megawatts, and most coal plants are in the range of hundreds of megawatts.)
Baseload plants are meant to run at 100 percent power, for weeks or months at a time, or in the case of nuclear plants, even years at a time. To meet the difference between baseload and peak (which can easily be 50 or 60 percent), generators that are more able to ramp up and down are called in and may shut down entirely in some periods.
While today’s nuclear reactors work very well at baseload generation, tomorrow’s are intended to be more flexible, which will be essential on a grid heavy with solar and wind. Solar and wind are energy sources that supporters call “variable” and detractors call “intermittent.” Whatever you call them, if we’re going to get to zero emissions, or near it, nuclear energy is going to have to perform flexibly to complement them.
A new generation of reactors is aiming to do just that.
Baseload isn’t enough
“Baseload” isn’t a virtue, it’s an attribute. It means it’s meant to run around the clock, compared to a “peaker” plant, which may run only a few hundred hours a year, only when demand is high, or a “mid-merit” plant, that may run for thousands of hours a year, but not continuously, or not at full power all the time.
A baseload plant isn’t better than a peaker plant any more than a mid-sized sedan is better than a U-Haul truck. They have different functions.
But in New England and around the country, the plants that meet variable demand are generally running on gas, and that’s not compatible with a completely clean system.
Nuclear plants don’t emit carbon dioxide when they run, but there are two problems with running them at less than full power, one financial and one engineering.
First, the engineering problem:
Today’s reactors are not a great way to deliver electricity on a variable basis. Most of the plants now in service are pressurized water reactors, whose output is cumbersome to adjust. For one thing, adjustments aren’t good for the fuel, which is ceramic uranium pellets inside metal tubes. Both of those expand when hot, but not quite at the same rate. When operators change the heat output of the fuel pellets, this changes the pellets’ interaction with the fuel cladding, and can cause fuel damage. Engineers spend extensive efforts studying the effects of “ramping” power levels on the fuel/cladding interaction.
And the operators of these reactors sometimes adjust the power by adding boric acid (yes, the same stuff used for eyewash) to the water, because the boron absorbs neutrons, stealing them from the chain reaction. Re-adjusting the boron concentration to return to full power is a slow process, and the water that is removed must be processed.
Boiling water reactors are a little easier to adjust, because they have a neat trick. In today’s reactors, called light-water reactors, the water does two jobs. One is to take heat from fission and move it to where it can be turned into mechanical power, by spinning a turbine, and then into electricity. The other is to slow down the neutrons, the sub-atomic particles that are emitted when uranium is split, so they are at an optimum speed for splitting the next uranium atom they encounter.
Water is a good moderator, but steam isn’t. Boiling water reactors, as the name implies, boil water in the core. The operators can vary the mixture of water and steam, to reduce output slightly.
Operators can also insert control rods. But that makes it harder to use up all the fuel, because when the time for refueling comes, some of the uranium will have been shielded from neutrons by the control rods and will not be fissioned.
Newer designs (including the new Vogtle units in Georgia) have a set of control rods called “gray rods” that block some of the neutrons but not all. That makes it easier to moderate the heat output and change the reactor’s energy output quickly. France, with an extremely high proportion of nuclear in its electricity mix, makes extensive use of that technique.
The second problem with ramping is economic. Nuclear plants cost a lot to build and running them economically means spreading that cost over as many kilowatt-hours of production as possible. But a zero-carbon system may have to include some scaling back of nuclear generation during peak hours of sun or wind.
That reduces a key metric called capacity factor, which is the fraction of energy produced compared to energy that would be made if a plant ran full out, 24/7/365.
Capacity factor is crucial to nuclear plants, and improvement to record levels (now more than 92 percent) are a key element in keeping them economically competitive. New reactors are likely to be more expensive, per kilowatt of capacity, than other technologies, so keeping them running at a high capacity factor will be critical too. Plants powered by natural gas can afford to run fewer hours, because they are cheaper to build, and because they save money by not burning fuel when they’re not operating. (Today’s nuclear plants, in contrast, are batch-loaded, like the batteries in a flashlight, and tend to replace the fuel on a fixed schedule, whether it’s used up or not. Some advanced reactors can refuel while running, so shutting down in slack periods would at least save them some fuel.)
There is another problem with baseload plants: baseload is becoming less predictable. In some cases, a sudden late-night surge of wind power will push supply above demand, and some baseload plants will have to back off.
How can advanced nuclear help?
The project that broke ground on June 10 in Kemmerer, Wyoming, near a retiring coal plant, is widely described as an advanced nuclear reactor. That’s true, but it misses half the point. The Natrium project, and other new reactors, are getting set up to do something that today’s reactors find very difficult: operate flexibly, in response to changing grid conditions, and, in some cases, store large amounts of energy.
The reactor produces enough heat to make a steady 345 megawatts of electricity, but the battery stores enough energy to allow production of up to 500 megawatts, for five and a half hours, meaning that it can store about 850 megawatt-hours. The obverse is also true: it can cut its output when there is a surfeit of intermittent renewable energy. In fact, it has to cut its output if it is going to store up heat for peak periods. It can go down to 100 megawatts. What makes up the difference is the temperature of the salt in the storage tank, which can vary from 238 degrees C to 621 degrees C. (For troglodytes and Americans, that’s 460 degrees to 1150 degrees F.) The salt is a known quantity; it’s already used in solar thermal plants.
And Natrium can change its output at 8 percent per minute.
Natrium’s isn’t the biggest battery in the world; there’s a lithium-ion plant in California that is 3,287 megawatts. But the Natrium plant, the first of a kind, has a modular design. Future units could add additional tanks
In California, for example, wholesale price swings of 9 cents for the price of a kwh over the course of a day are common. (For comparison, the wholesale price of electricity for the whole country outside the northeast is usually between 3 and 4 cents per kilowatt-hour. It’s close to 5 cents in New York and 6 cents in New England. Essentially this means that the swing in California prices is substantially larger than the total cost in the rest of the country.
Being able to sell more in high-price periods and less in low-price periods can make a generator much more profitable. When solar quits at sunset but people are arriving home from work and switching on microwaves, air conditioners and big-screen TVs, and recharging their electric vehicles, megawatt-hours can sell at prices resembling black-market Cabbage Patch dolls or Rolling Stones tickets.
The problem is illustrated by a graph of demand in California now known as the “duck curve.” Squint and you can see the outline of a duck. The curve shows power load minus solar, and at noon on a day with light demand, all the system’s needs are met by solar. The problem is that at sunset, solar disappears fast and demand soars.
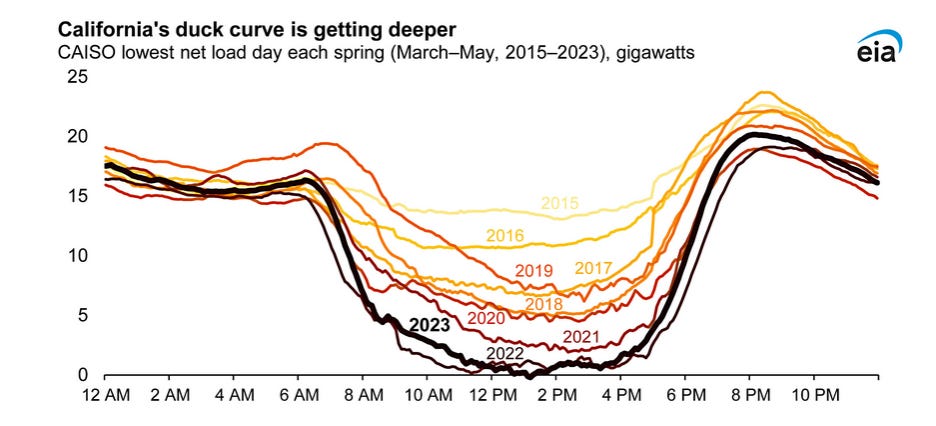
There are other forms of storage, of course, but they have strong environmental drawbacks. Lithium-ion batteries require extensive mining and energy-intensive processing to build. Pumped hydro creates two mostly-sterile reservoirs, one the uphill side and one on the downhill. (Fish, plants and other lake life don’t do well in places where the water levels rise and fall a lot, frequently.)
And storage isn’t inherently clean; in fact, it tends to increase the carbon footprint. Historically, utilities have used it to meet peak demand, but they’ve charged it up at night by burning extra fossil fuel, usually coal. And the oldest form of big energy storage, pumped hydro, requires three kilowatt-hours of input for every two kilowatt-hours it gives back. So, energy from a coal plant starts out with a carbon footprint of 1.14 pounds (The U.S. average), but if it goes through a pumped storage plant before it reaches consumers, it would be 1.71 pounds, not counting the carbon emissions from the upper and lower reservoirs of the pumped storage system.[MW1] [MW2]
The parent company of Detroit Edison, DTE Energy, announced recently that it would preplace a coal-powered plant with a 220-megawatt, 880-megawatt-hour battery installation, which would be the largest battery storage system in the Great Lakes. The company said it would make the system cleaner but did not identify the source of the energy that would be used to charge it. (DTE does plan to add more renewables, however.)
A nuclear plant storage system, in contrast, is charged with carbon-free energy.
If Reactors Don’t Store Energy, They Can At Least Be Flexible
NuScale Power is another example of flexibility. It has fuel like today’s pressurized water reactors, but it can do things those reactors can’t. For one, it can divert some, or all, of the steam from one of its 77-megawatt modules away from the turbine and straight to the condenser, where the steam is turned back into water for re-heating. That gives nearly instant flexibility. Over time, it can reduce power in a module, or, if located in a place with other carbon-free resources that are seasonal in nature, like hydroelectric energy, modules can be shut down completely.
NuScale performed a study of the problem, taking a year of actual production data from a 58-megawatt wind farm in Utah called Horse Butte, and marrying it, on paper at least, to a hypothetical NuScale module. The module could increase and decrease its output fast enough to provide any level of power desired, in combination with the wind farm.
Other designs use “pebbles,” kernels of fuel wrapped in layers of heat-resistant materials. Kairos Power and X-energy, the first cooled by molten salt and the second by inert gas, are in this category. The pebbles can handle power level changes.
Other designs use fuel dissolved in molten salt, which can handle a wide range of temperatures in normal operation.
What’s Ahead?
There are other concepts; during periods of low electrical demand, a reactor’s energy could be used to split water molecules into oxygen and hydrogen. The hydrogen can be turned back into electricity later, without carbon emissions, by burning it or running it through a fuel cell. But the equipment to split water molecules is expensive, and the economics work best at a high capacity factor.
A reactor could also be used to desalinate water during off hours. Water is easy to store, and the precise hour of its production isn’t important for a thirsty city trying to fill a reservoir. Electricity is the opposite case; it’s a just-in-time product.
A system that uses a lot of wind and solar will need a lot of storage. And it will need nimble partners; the reactors’ role may resemble the old joke about Ginger Rogers, who had to do all the dance steps that her partner Fred Astaire did, except backwards and in high heels. Flexible reactors, and reactors that can store their output, are good dance partners for wind and solar; in fact, they could increase the amount of wind and solar that the grid can integrate.
System planners recognize the value of flexible generation. Policy makers would do well to appreciate the abilities of an emerging generation of advanced reactors to operate that way too.